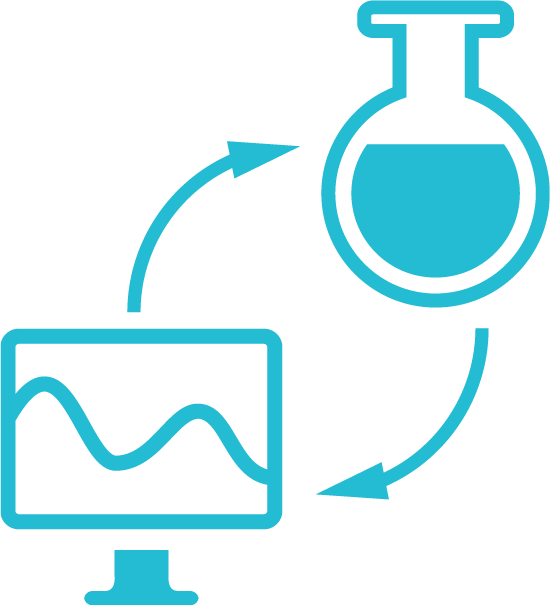
Thrust 3: Exploring Mesophase Space
Assembly using crystallization approaches has beautifully demonstrated the ability to generate equilibrium, densest-packed architectures with a variety of building blocks—from NC Bravais superlattices to protein MOFs—and will be used to characterize the programmed building-block interactions that result from nanosynthesis efforts in Thrust 2. Characterization of these crystallization experiments will be carried out by Tezcan, Tao, and Figueroa via electron and optical microscopy, SAXS/GISAXS/WAXS, and the high-resolution methods described in Thrust 4. A vast range of phase space associated with low-density architectures remains unexplored, resulting in a persistent, large void in materials discovery since mesophases that occur at low or intermediate volume fractions (rather than densest-packed configurations) are most likely to generate functional materials of interest, including the responsive and porous materials explored by IRG1. For example, Tezcan’s group recently pioneered the synthesis of protein hydrogels. Such materials present emergent and even anomalous materials structure-function properties, since even the most dynamic porous materials tend to be brittle and isolated as microcrystalline powders.
Thrust 3’s computational efforts will identify phases and phase transitions (e.g., disorder-to-order) for NC and protein assemblies. Possible ordered phases include crystalline lattices, quasi-crystals, or mesophases that possess varying degrees of structural order, such as liquid-crystalline phases (i.e., orientational order) and plastic-crystalline phases (i.e., translational order). These mesophases are predicted to occur over long time and length scales relative to “fast” crystallization events characteristic of the molecular scale. While CG models can be further coarse-grained to enable access to the millisecond timescale and micrometer length scale, two-body free-energy landscapes may not provide all of the required information for prediction of mesoscale phases. Moreover, as detailed previously, multibody interactions are often critical determinants in assembly structures.
Thrust 3 will explore mesoscale assembly by studying a large number (20-50) of particles using the CG models in Thrust 1. One possible approach for simulating large ensembles of particles is to carry out brute-force MD simulations, with the inter-particle interactions described with a low-resolution CG model. However, such an approach may still be computationally prohibitive and may not be truly representative of the experimental system. Thus, to accelerate the process of generating mesophase diagrams, Arya will formulate analytical scaling expressions for the interparticle free energies based on colloid and polymer physics, as done recently for the case of polymer-grafted nanocubes. Two computational strategies will be employed. The first involves the use of powerful optimization algorithms that combine conjugate gradient, transition-state sampling, basin-hopping MC, and clustering algorithms to rapidly locate the globally stable configuration for a large number of particles. The second strategy uses explicit determination of the free-energy differences between candidate mesophases and interpolation techniques to generate a complete free-energy landscape. Here, we will calculate the free-energy difference between all pairs of candidates via thermodynamic integration and by picking the mesophase with the lowest free energy.
Mesophase diagrams developed by Arya will provide a prediction for mesoscale assembly experiments, including the response of the system to changing experimental conditions such as temperature and pressure. A key technique that will be used to fabricate these low-density mesophases is Langmuir-Blodgett (LB) assembly. LB assembly involves isothermal compression of building blocks at an air-water interface, which was pioneered for nanostructure assembly by Tao. Using this technique, Tao will use the NC and protein building blocks synthesized in Thrust 1 to experimentally measure equations of state for 2D assemblies. The LB technique is particularly well suited for probing dynamic mesophase transitions and non-equilibrium metastable mesophases, since compression is used to impart very small pressure changes at the air-water interface. Experimental results from Tao will be used to validate mesophase predictions from Arya by demonstrating a given mesophase (e.g., a plastic rotator phase) for a specific building block, temperature, and pressure. If LB experiments do not agree with mesophase predictions, it suggests that emergent multi-body effects are not being appropriately captured by our model, and we will re-evaluate the resolution of the CG model.
Tao and Fraño will couple assembly experiments and in situ characterization techniques—including in situ small-angle X-ray scattering (SAXS) and X-ray photon correlation spectroscopy (XPCS)—to probe particle dynamics at the nano- to microscale and over time scales of minutes to hours. Unlike conventional X-ray scattering experiments that provide globally averaged order parameters, XPCS takes advantage of the coherent nature of scattered X-rays, which yield speckle patterns. These patterns are a Fourier-space representation of how scatterers are distributed over the spatial footprint (>10 μm) of the beam but are derived from scattering off Bragg planes (atomic resolution). In the context of IRG1, XPCS is perhaps the only technique capable of yielding dynamic structural information spanning atomic to mesoscopic length scales. XPCS experiments will take place at national laboratory synchrotron facilities, which are now capable of yielding X-ray beams with a high coherent flux. In this effort, Tao and Fraño will partner with the Van Buuren group at LLNL. Van Buuren has a long-standing interest in understanding the fundamental structure and interactions of materials at the nanoscale, and they have developed best-in-class X-ray and synchrotron tools for probing and understanding materials phenomena. We will leverage LLNL’s expertise in X-ray characterization techniques including diffractive imaging, high resolution tomography and SAXS to visualize diffusion, positioning, structure, and function.